Revised 2023
HISTORY
The concept of interlocking concrete pavement dates back to the roads of the Roman Empire. See Figure 1. They were constructed with tightlyfitted stone paving units set on a compacted aggregate base. The modern version, concrete pavers, is manufactured with tight tolerances to help ensure interlock. Concrete pavers were developed in the Netherlands in the late 1940s as a replacement for clay brick streets. A strong, millennia-old tradition of segmental paving in Europe enabled interlocking concrete pavement to spread quickly. It is now established as a conventional means of paving there with some four billion ft2 (400 million m2) installed annually. Concrete pavers came to North America in the 1970s. They have been used successfully in numerous residential, commercial, municipal, port and airport applications. This Tech Note covers the structural design of interlocking concrete pavement over an aggregate base as well as asphalt and cement stabilized bases, asphalt concrete and Portland cement concrete bases.
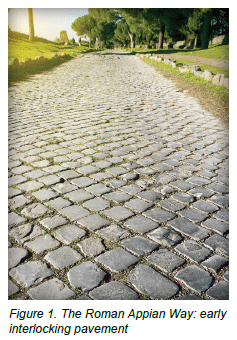
ADVANTAGES
The paving system offers the advantages of concrete materials and flexible asphalt pavement. As high-strength concrete, the units have high resistance to freeze-thaw cycles and deicing salts, high abrasion and skid resistance, no damage from petroleum products or indentations from high temperatures. Once installed, there is no waiting time for curing. The pavement is immediately ready for traffic. Cracking and degradation of the surface is minimized because of the numerous sand-filled joints which act as a means for load transfer without damaging the pavement surface. Like flexible asphalt pavement, an aggregate base accommodates minor settlement without surface cracking. An aggregate base facilitates fast construction, as well as access to underground utilities. Mechanical installation of concrete pavers can further reduce construction time and costs. Pavement reinstatement is enhanced by reusing paving units, thereby minimizing costs and reducing waste.
THE PRINCIPLE OF INTERLOCK
Interlock is the inability of a paver to move independently from its neighbors. It is critical to the structural performance of interlocking concrete pavement. When considering design and construction, three types of interlock must be achieved: vertical, rotational, and horizontal interlock. These are illustrated in Figure 2. Vertical interlock is achieved by shear transfer of loads to surrounding units through sand in the joints. Rotational interlock is maintained by the pavers being of sufficient thickness, meeting recommended plan and aspect ratios, placed closely together, and restrained by a curb from lateral forces of vehicle tires. Rotational interlock can be further enhanced if there is a slight crown to the pavement cross section. Besides facilitating drainage, the crown enables the pavement surface to stiffen and further lock up as the pavement undergoes vehicle loading due to traffic.
Horizontal interlock is primarily achieved through the use of laying patterns that disperse forces from braking, turning and accelerating vehicles. Herringbone patterns are the most effective laying patterns for maintaining interlock (see Figure 3). Testing has shown that these patterns offer greater structural capacity and resistance to lateral movement than other laying patterns (Shackel 1979 and 1980). Therefore, herringbone patterns are recommended in areas subject to vehicular traffic. See Figure 3. Stable edge restraints such as curbs are essential. They provide better horizontal interlock among the units while they are subject to repeated lateral loads from vehicle tires. Tech Note PAV-TEC-003–Edge Restraints for Interlocking Concrete Pavements offers guidance on the selection and detailing of edge restraints for a range of applications.
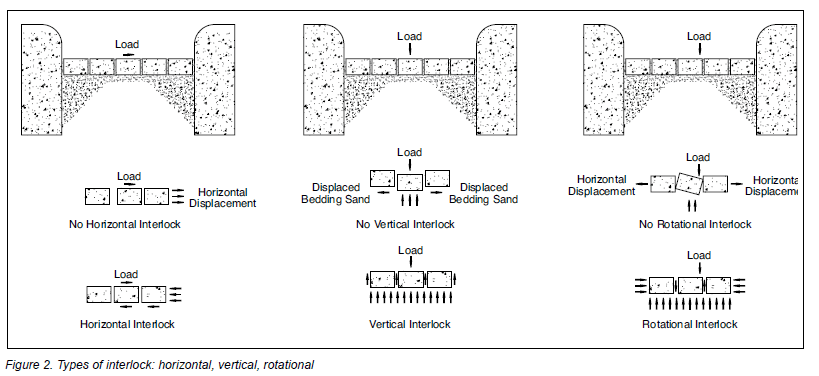
TYPICAL PAVEMENT DESIGN AND CONSTRUCTION
Flexible pavement design uses untreated aggregate, cement- or asphalt-treated aggregates or asphalt under the concrete pavers and bedding layer. Flexible pavements distribute the loads to the subgrade by spreading them through consecutively weaker layers to the compacted soil subgrade. Such pavements are often preferred in colder climates because they can offer greater protection against frost heaving. Figure 4 illustrates typical schematic cross sections for interlocking concrete pavement designed as a flexible system. The base and subbase are compacted aggregate. Some road agencies may use opengraded drainage bases as well. Many pavements for city and residential uses do not require an aggregate subbase except for very heavy use or over a weak soil subgrade. In these situations it may be more economical to use asphalt or cementstabilized base layers. They are often placed over a subbase layer of unbound compacted aggregate and cement-stabilized soil offers another option for improving structural capacity.
Construction is covered in Tech Note PAV-TEC-002Construction of Interlocking Concrete Pavement. The steps for preparing the soil subgrade and base materials are similar to those required for flexible asphalt pavements. After the base surface is built to specified elevations and surface tolerances, bedding sand is screeded in an even layer, typically 1 in. (25 mm) thick. The units are placed, manually or mechanically, on the even bedding sand constrained by stationary edge restraints. Slopes normally should be a minimum of 1.5%. In the case of roads, the minimum longitudinal slope should be 1% with a minimum cross slope of 2%.
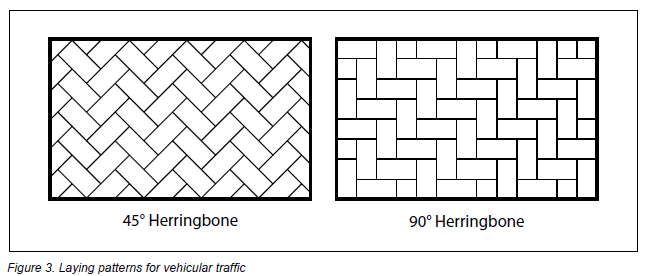
The pavers are vibrated with a minimum 5,000 lbf (22 kN), high frequency plate compactor. This action forces sand into the bottom of the joints of the pavers and begins compaction of the bedding sand. Sand is then spread and swept into the joints, and the process repeated until the joints are filled. Complete compaction of the joint sand and slight settlement of the pavers tightens them. During compaction, the pavement is transformed from a loose collection of pavers to an interlocking system capable of spreading vertical loads horizontally. This occurs through shear forces in the joints.
STRUCTURAL DESIGN PROCEDURE
The load distribution and failure modes of flexible asphalt and interlocking concrete pavement are very similar: permanent deformation from repetitive loads. Since failure modes are similar, flexible pavement design procedures are used. The structural design procedures are for roads and parking lots. Base design for crosswalks should consider using stablized aggregate or cast-in-place concrete with sand-set paving units, or bitumen-set paving units over concrete. Additonal information on crosswalk design and bitumen-set applications can be found in the following two Tech Notes: Tech Note PAV-TEC-019–Design, Construction and Maintenance of Interlocking Concrete Pavement Crosswalks and Tech Note PAV-TEC-020 –Construction of Bituminous-Sand Set Interlocking Concrete Pavement. Stiffer bases will compensate for stress concentration on the subgrade and base where the pavers meet adjoining pavement materials. Design for heavy duty pavements such as port and airport pavements is covered in CMHA manuals entitled, Port and Industrial Pavement Design for Concrete Pavers and Airfield Pavement Design with Concrete Pavers.
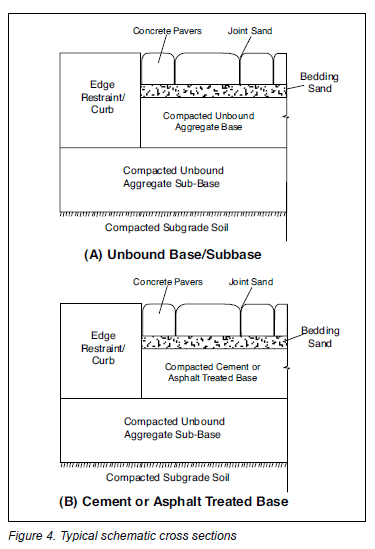
DESIGN METHODOLOGY
Structural design of interlocking concrete pavements follows the American Society of Civil Engineers Transportation & Development Institute standard (ASCE/T&DI 58-16), Structural Design of Interlocking Concrete Pavement for Municipal Streets and Roadways (ASCE 2016). This standard applies to paved areas subject to applicable permitted axle loads and trafficked up to 10 million (18,000 lb or 80 kN) equivalent single axle loads (ESALs) with a vehicle speed of up to 45 mph (70 km/h). The standard provides information required for design, key design elements, design tables for pavement equivalent structural design, construction considerations, applicable standards, definitions and best practices. Readers are encouraged to purchase and review this guideline standard.
The ASCE standard relies on the flexible pavement design method described in the 1993 Guide for Design of Pavement Structures published by the American Association of State Highway and Transportation Officials (AASHTO 1993). Future versions of the ASCE standard may include the mechanisticempirical design methodology as described in the 2004 Guide for Mechanistic Empirical Design of New and Rehabilitated Pavement Structures (AASHTO 2004). The level of detailed information required to use this procedure is unavailable for most non-highway applications.
The design process is characterized by the flowchart shown in Figure 5. The following provides information on the key input variables noted in the flowchart.
Design Traffic—When pavement is trafficked, it receives wear or damage usually evidenced as the depth of rutting in flexible asphalt pavements and the extent of cracking in rigid concrete pavements. For interlocking concrete pavements, damage is typically measured by the depth of rutting since it behaves as a flexible pavement similar to asphalt. Cracked paving units are rarely evidence of a pavement damaged by traffic loads and therefore are not typically used as a means to estimate damage or wear of an interlocking concrete pavement.
As with all pavements, the amount of damage from traffic depends on the weight of the vehicles and the number of expected passes over a given period of time. The period of time, or design life, is 20 to 40 years. Design life is the period of time a pavement will last before damage requires major rehabilitation, often complete removal and replacement. The designer or transportation agency selects a design life in years which is influenced by the available budget to construct or rehabilitate a pavement.
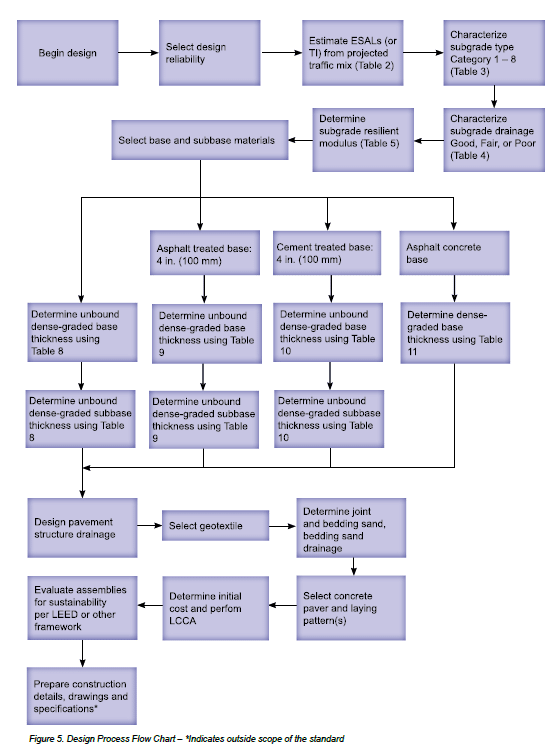
Predicting traffic over the life of the pavement is an estimate of various vehicle loads, axle and wheel configurations, and the number of loads (repetitions). The actual amount of traffic loads can often exceed the predicted loads. Therefore, engineering judgment is required in estimating expected sources of traffic and loads well into the future. When future traffic loads are difficult to predict, an engineer will often design a pavement for higher loads to ensure that the risk of excessive pavement damage is low over the service life of the pavement.
Compared to cars, trucks and busses do the most damage to pavements because their wheel loads are much higher than cars. One pass of a fully loaded truck will exert more damage to pavement than several thousand cars passing over it. Since there is a range of expected loads (usually expressed as axle loads) over a pavement during its life, AASHTO developed a means to normalize or equalize all axle loads of them into a single axle load exerted repeatedly over the life of the pavement.
The 1993 AASHTO Guide characterizes traffic loads as the number of 80 kN or 18,000 lbs equivalent single axle loads or ESALs. The 18,000 lbs (80 kN) load emerged from AASHTO (then called AASHO) road tests conducted in the 1950s and have remained as a convenient means to quantify a range of different vehicle axle loads. The AASHTO tests demonstrated that loads and resulting damage to pavement is not linear but exponential as loads increase. The tests showed that for every incremental increase in axle load, damage to the pavement increased by roughly the fourth power. This exponential loaddamage relationship resulted in determining ESALs by taking the weight of each axle and dividing each by a ‘standard’ ESAL of 18,000 lbs or 80 kN. Then the quotient is raised to the fourth power.
For example, a five axle tractor-trailer truck has two rear axles on the trailer each exerting 18,000 lbs or 80 kN; two on the back of the truck at 15,800 lbs or 70 kN; and one in the front (steering) at 11,000 lbs or 50 kN. AASHTO uses the following relationships called load equivalency factors or LEFs for each axle to estimate ESALs. These express the exponential relationship between damage and loads. LEF and ESALs for this truck are as follows:
Trailer: LEF = (80/80)4 = 1 (x 2 axles) = 2 ESALs
Truck rear: LEF= (70/80)4 = 0.6 (x 2 axles) = 1.2 ESALs
Truck front: LEF = (50/80)4 = 0.15 ESALs
When added together, all LEFs = 3.35 ESALs. So for every pass across a pavement, this truck exerts 3.35 80 kN (18,000 lbs) ESALs.
To put automobile axle loads into perspective, the axle loads of one passenger car placed into the formula yields about 0.0002 ESALs. Therefore, pavement design primarily considers trucks and busses because they exert the highest loads and most damage. In contrast, thousands of cars are required to apply the same loading and damage as one passage of a truck.
The more axles on trucks the better, since tandem axles spread loads over a wider area and render lower damage for each pass of the vehicle over a pavement. Another way to illustrate this is one single axle load of 36,000 lbs (160 kN) exerts the same damage as 16 passes of a single axle load of 18,000 lbs (80 kN) or (36/18)4 = 16. Therefore, doubling the axle load increases the damage 16 times.
The California Department of Transportation or Caltrans uses Traffic Index or TI rather than ESALs. Converting ESALs to TI is accomplished by using the formula below. Table 1 illustrates the relationships between ESALs and TIs. Table 2 provides AASHTO road classifications and typical lifetime ESALs and TIs.
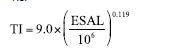
For the ASCE standard, ESAL levels are provided for 10 typical levels of municipal traffic up to a maximum of 10 million ESALs. The designer needs to select the appropriate traffic level and design life. The typical initial design life for municipal pavements is on the order of 20 to 40 years.
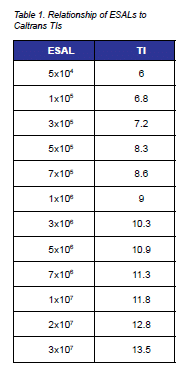
Subgrade Characterization—The next step is for the designer to characterize the subgrade soil and drainage for the purpose of selecting a subgrade strength. Typically the resilient modulus or Mr (AASHTO T-307) is used to describe the strength of the subgrade soil. This can be determined directly from laboratory testing. Other means to characterize soil strength include California Bearing Ratio or CBR (ASTM D1883) and R-value (ASTM D2844) tests. The relationship among Mr, CBR and R-value of subgrade soils are characterized by the equations below:
Mr in psi = 2,555 * (CBR)0.64
Mr in MPa = 17.61 x CBR0.64
Mr in psi = 1,155 + 555 x R
Mr in MPa = (1,155 + 555 x R)/145
The ASCE standard utilizes eight categories of subgrade quality ranging from good quality gravels and rock with excellent drainage to poor quality clay materials that are semi-impervious to water. Subgrade types are classified according to the Unified Soils Classification method (ASTM D2487). Soil categories in Table 3 are from the standard and are provided to the user for guidance only. Actual laboratory characterization of subgrade properties for each project is recommended. Designers are cautioned against making generalizations.
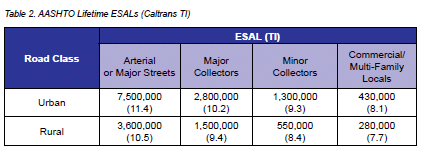
Once the general subgrade type has been selected, then the drainage quality of the subgrade and pavement structure is characterized (See Table 4). Depending on the type of subgrade, the strength of the pavement may be reduced if there is excess water in the subgrade. The standard includes an adjustment to the resilient modulus of the subgrade based on the overall quality of the pavement drainage, as shown in Table 5.
Selection of Base Material—The next step in the design process is selecting the type of base material for the pavement. The standard supports the use of bound and unbound bases.
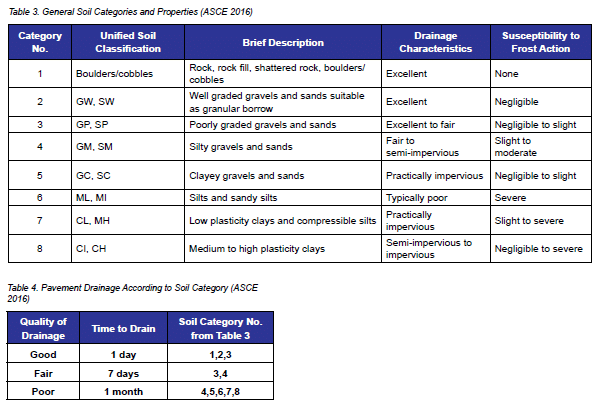
For unbound dense-graded bases, the aggregates are required to be crushed, angular materials. Crushed aggregate bases used in highway construction are generally suitable for interlocking concrete pavement, and unbound base materials should meet the local state, provincial or municipal standards governing base materials.
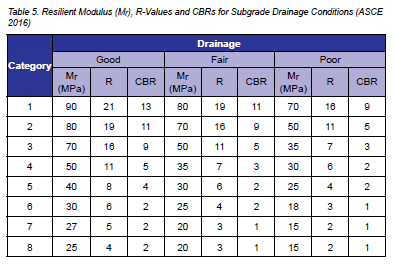
Where local specifications are unavailable, the base material is required to meet the gradation requirements according to ASTM D 2940. Table 6 includes these requirements. The minimum required strength of the unbound base is a CBR of 80% or equivalent bearing strength as described by the test methods in Section 3.6 of the standard. Unbound base materials are required to have a maximum loss of 60% when tested in accordance with CSA A23.2-29A (MicroDeval abrasion) and a maximum loss of 40% when tested in accordance with ASTM C 131 or CSA A23.2-17A (Los Angeles abrasion).
The required plasticity index is a maximum of 6 and the maximum liquid limit of 25 when tested in accordance with ASTM D4318 and AASHTO T-89 and T-90. For constructability purposes, the minimum design unbound base thickness is 4 in. (100 mm) for traffic less than 500,000 ESALs and 6 in. (150 mm) for 500,000 or higher ESALs. Figure 6 illustrates a typical cross section with an unstabilized, dense-graded base.
For bound or treated bases, asphalt-treated base (ATB) and cement-treated base (CTB) materials and installation are required to conform to provincial, state or local specifications for a dense-graded, compacted, asphalt concrete. ATB material is required to have a minimum Marshall stability of 1,800 lbf (8000 N) per ASTM D5 or AASHTO T-49. Use of the appropriate asphalt (performance grade) binder for local climate conditions is also recommended. For example, a state department of transportation Superpave intermediate binder course mix required for interstate or primary roads may be adequate. Cement-treated base material is required to have a minimum 7-day unconfined compressive strength of 650 psi (4.5 MPa) per ASTM D4320 and D4219. For constructability purposes, the minimum bound base thickness for design purposes is set at 4 in. (100 mm). Figure 7 illustrates a typical cross section with treated bases or an asphalt base and drainage holes.
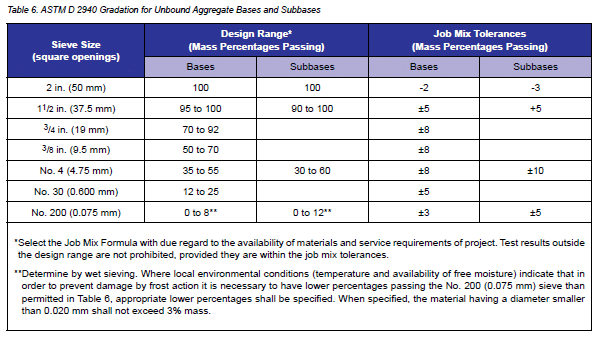
Asphalt bases should conform to typical provincial, state or municipal material and construction specifications for asphalt pavements. This layer does not require a surface riding layer of fine aggregate and consists of coarser aggregates and asphalt cement. The asphalt base layer thicknesses noted in Table 11 vary between 2 in. (50 mm) and 8.5 in. (220 mm) depending on traffic, soil category and drainage conditions.
Subbase Materials—Aggregates for subbase are crushed, angular materials typically used in highway construction are generally suitable for interlocking concrete pavement. All bound or treated bases are constructed over 4 to 8 in. (100 to 200 mm) unbound dense-graded aggregate base as described above. Unbound subbase materials are required to meet the local state, provincial or municipal standards governing subbase materials. Local road agencies may also use opengraded subbases for drainage. Where local specifications are unavailable, the subbase is required to meet the gradation requirements according to ASTM D2940 noted in Table 6. The required minimum strength of the unbound subbase is a CBR of 40% per ASTM D1883. The required plasticity index is a maximum of 10 and the maximum liquid limit of 25 according to ASTM D4318 and AASHTO T-90.
Compaction Requirements—Compaction of the subgrade soil during con struction should be at least 95% Standard Proctor Density as tested using AASHTO T-99 or ASTM D698 for cohesive (clay) soils and at least 95% Modified Proctor density as tested using AASHTO T-180 or ASTM D1557 for cohesionless (sandy and gravelly) soils. The higher compaction standards described in T-180 or D 1557 are preferred. The effective depth of compaction for all cases should be at least the top 12 in. (300 mm). Soils having an Mr of 4,500 psi (31 MPa) or less (CBR of 3% or less or R value of 8 or less) should be evaluated for replacement with a higher bearing strength material, installation of an aggregate subbase capping layer, improvement by cement stabilization or use of geotextiles at the soil/subbase interface or geogrids. ATB and CTB density testing should conform to provincial, state or local requirements. In-place density testing of all of the soil subgrade and pavement layers should be included in the project construction specifications and documented with written testing reports. Density tests on the site project as part of construction quality control are critical to pavement performance. Difficult to compact areas can include areas next to curbs, other pavements, and around utility structures. Such areas may require additional compaction or use of manual equipment to achieve specified densities.
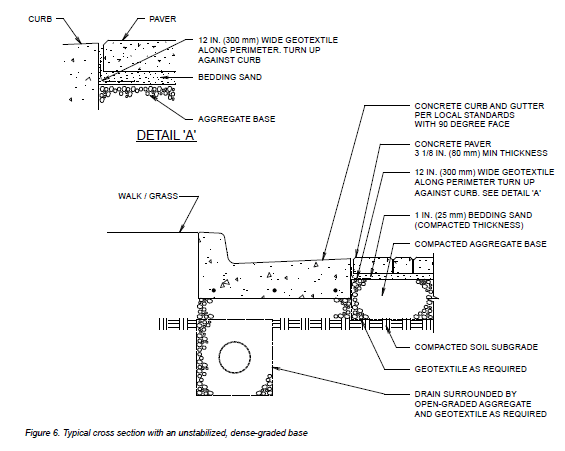
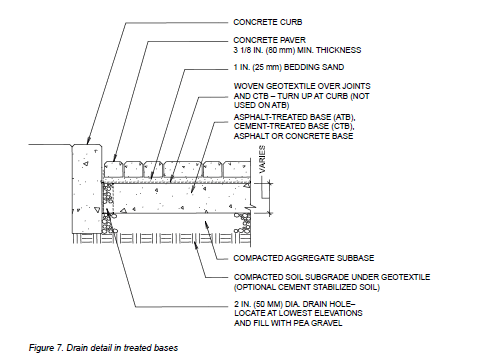
Structural Contribution of the Concrete Pavers and Bedding Sand Layer—Research using accelerated traffic studies and non-destructive structural testing in the United States and overseas has shown that the combined paver and sand layers stiffen while exposed to greater numbers of axle loads. The progressive stiffening that results in “lock up” generally occurs early in the life of the pavement, before 10,000 ESALs (Rada 1990). Once this number of loads has been applied, Mr = 450,000 psi (3,100 MPa) for the combined 31/8 in. (80 mm) thick paver and 1 in. (25 mm) of bedding sand. Pavement stiffening and stabilizing can be accelerated by static proof-rolling with an 8–10 ton (8–10 T) rubber tired roller.
The above resilient modulus is similar to that of an equivalent thickness of asphalt. The 31/8 in. (80 mm) thick pavers and 1 in. (25 mm) thick bedding sand together have an AASHTO layer coefficient at least equal to the same thickness of asphalt, i.e., 0.44 per inch (25 mm). This renders an AASHTO Structural Number or SN of 4.125 in. x 0.44 = 1.82 for this pavement layer. The recommended Caltrans Gravel Equivalency (GE) for the concrete paver layer = 2 and unlike asphalt the GE for concrete pavers does not decrease with increasing TIs. The modulus or stiffness of the concrete paver layer will not substantially decrease as temperature increases nor will they become brittle in cold climates. The surfacing can withstand loads without distress and deterioration in temperature extremes.
Bedding and Joint Sand Selection—Bedding sand thickness should be consistent throughout the pavement and not exceed 1 in. (25 mm) after compaction. A thicker sand layer will not provide stability. Very thin sand layers (less than 3/4 in. [20 mm] after compaction) may not produce the locking up action obtained by sand migration upward into the joints during the initial compaction in construction. The bedding layer should conform to the gradation in ASTM C 33, as shown in Table 7. Do not use screenings or stone dust. The sand should be as hard as practically available and the particle shape should be sub-angular. Tech Note PAV-TEC-017–Bedding Sand Selection for Interlocking Concrete Pavements in Vehicular Applications provides additional information on gradation and test criteria on selecting bedding sands for pavements subject to 1.5 million lifetime ESALs or higher.
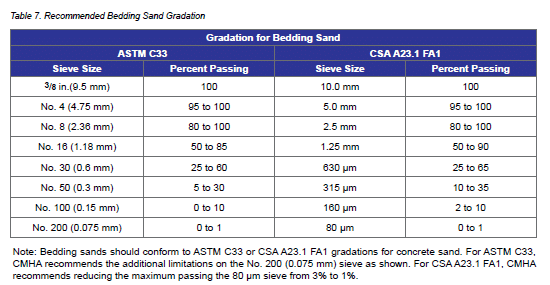
Joint sand provides vertical interlock and shear transfer of loads. It can be slightly finer than the bedding sand. Gradation for joint material should comply with ASTM C144 or CSA A179 with a maximum 100% passing the No. 16 (1.18 mm) sieve and no more than 5% passing the No. 200 (0.075 mm) sieve. Bedding sand may be used for joint sand. Additional effort in filling the joints during compaction may be required due to its coarser gradation.
Concrete Paver Selection—Concrete pavers shall meet the product requirements of ASTM C936 Standard Specification for Solid Interlocking Paving Units in the United States and CSA A231.2 Precast Concrete Pavers in Canada. For vehicular applications, the ASCE standard requires pavers that have an aspect ratio (overall length/thickness) less than or equal to 3:1 and a minimum thickness of 31/8 in. (80 mm). A 45 or 90-degree herringbone laying pattern is recommended with sailor courses at the perimeter. No less than one-third of a cut paver should be exposed to tire traffic. The designer is advised that alternative laying patterns may be considered as long as they are functionally and structurally equivalent. Other shapes than rectangular pavers can be considered in the design with the responsibility of the design engineer to confirm that the structural capacity is at least equal to the AASHTO structural number layer coefficient (SN) of the 0.44 for the pavers and bedding sand layer used in the standard, either by testing or confirmation from the manufacturer. CMHA takes a conservative approach by not recognizing differences among paver shapes with respect to structural and functional performance. Certain manufacturers may have materials and data that discuss the potential benefits of shapes on functional and structural performance in vehicular applications.
Subbase Thickness and Final Pavement Structural Design—The required subbase thickness is determined based on the design reliability, design life, estimated traffic, subgrade soil type, pavement structure drainage and base type selected. Subbase thicknesses are determined from one of the four design tables. The design tables provide structural design thicknesses primarily for unbound bases (granular base), ATB, and CTB. However, a thickness design table is also provided for asphalt concrete (AC) bases to reduce thick pavement structures associated with high traffic/low subgrade strength conditions. In the development of the AC table, an AASHTO structural layer coefficient of 0.44 has been assumed for AC. For AC layer coefficients other than 0.44, the designer is advised to consult the 1993 AASHTO Guide. Tables 8 through 11 show the design tables for unbound granular base, ATB, CTB and AC for 80% reliability factor using the 1993 AASHTO Guide. This reliability factor is slightly higher than the 75% in the ASCE Standard tables and in some cases can result in slightly thicker subbases, specifically in weak soils.
DESIGN EXAMPLE
Design examples are given with good soil conditions (subgrade category 4) and, fair drainage, with lifetime traffic of 5,000,000 ESALs. Designs developed for these conditions are shown in Table 12.
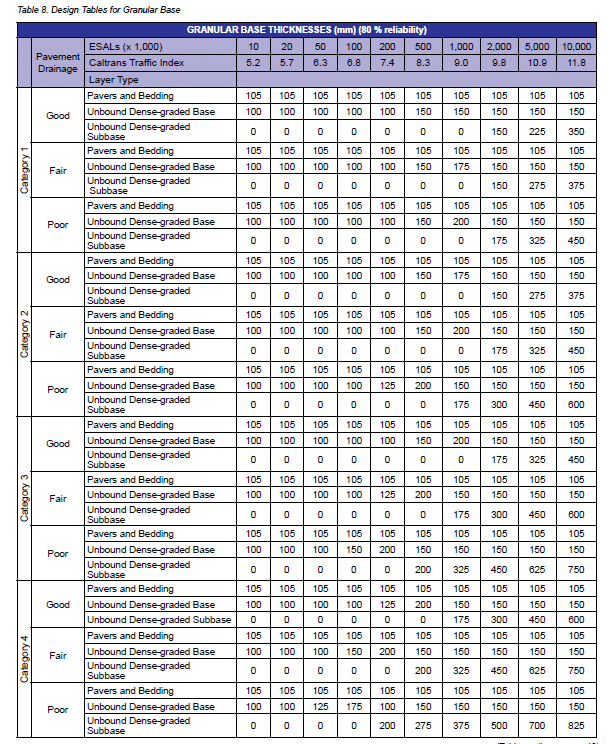
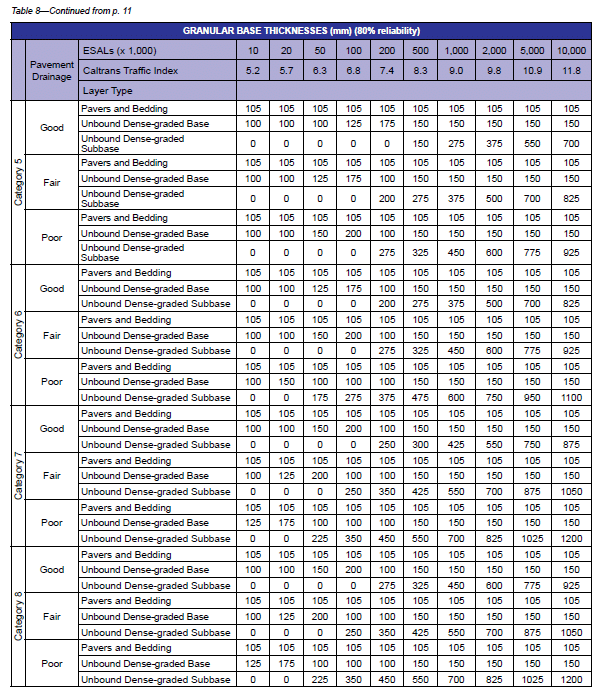
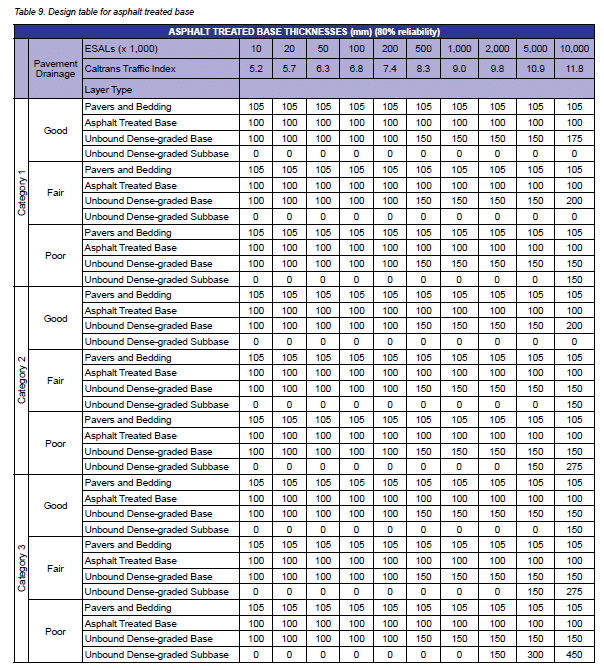
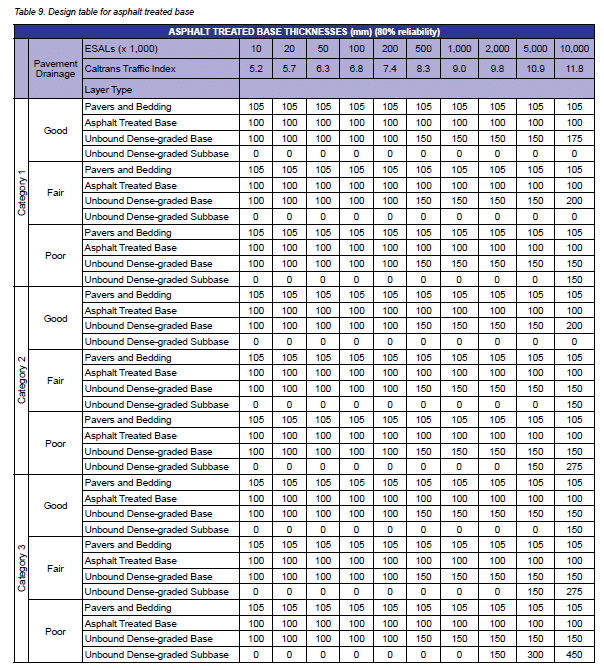
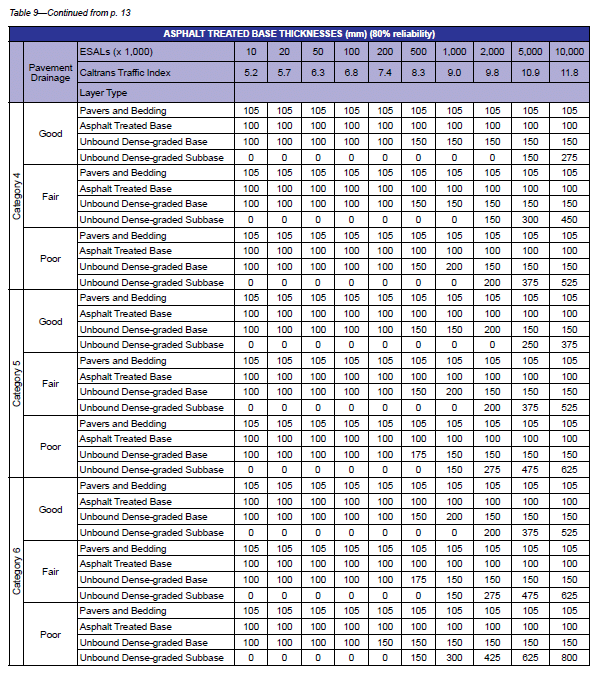
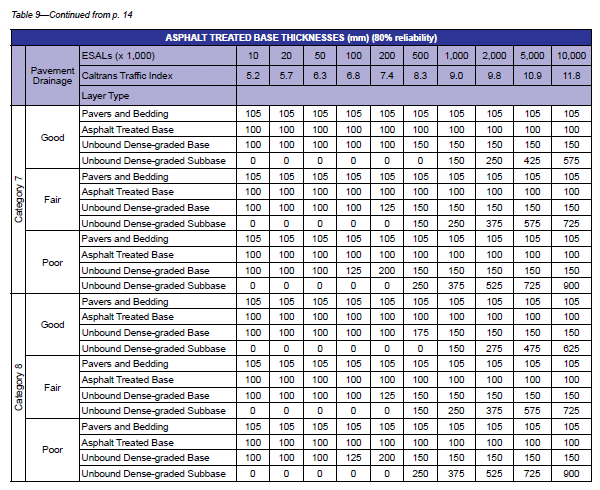
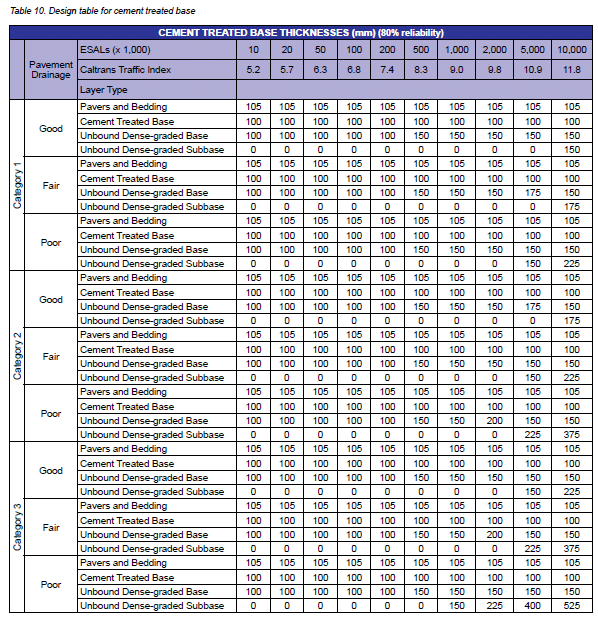
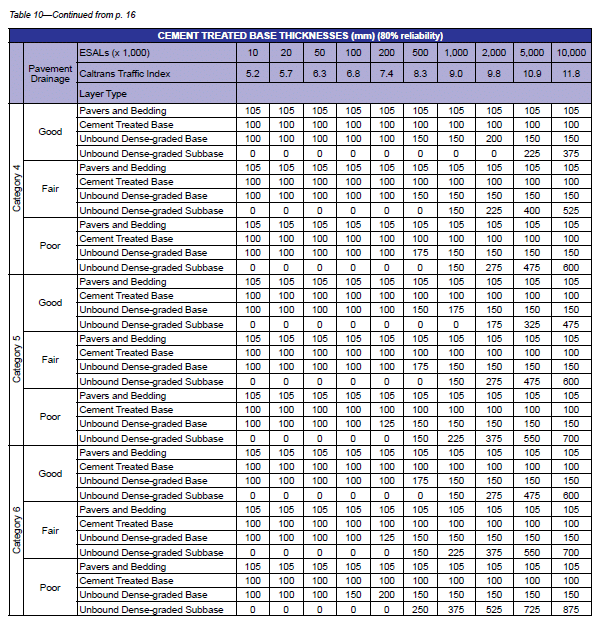
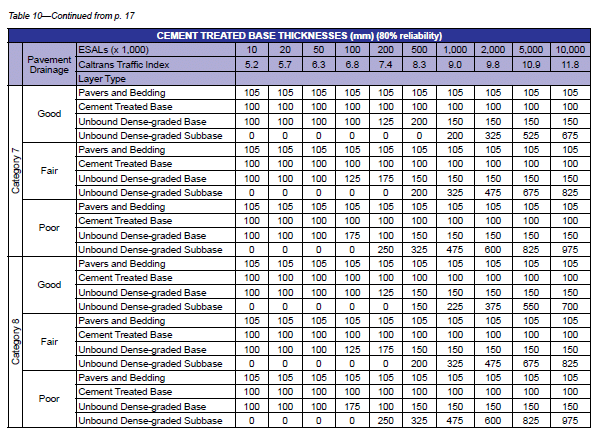
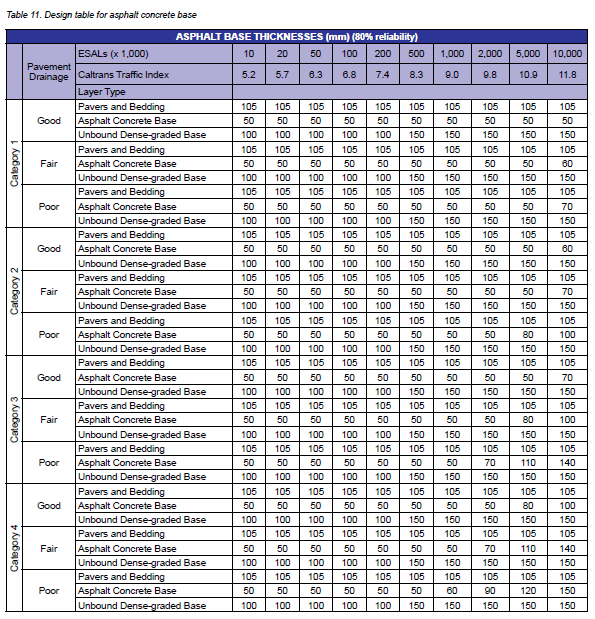
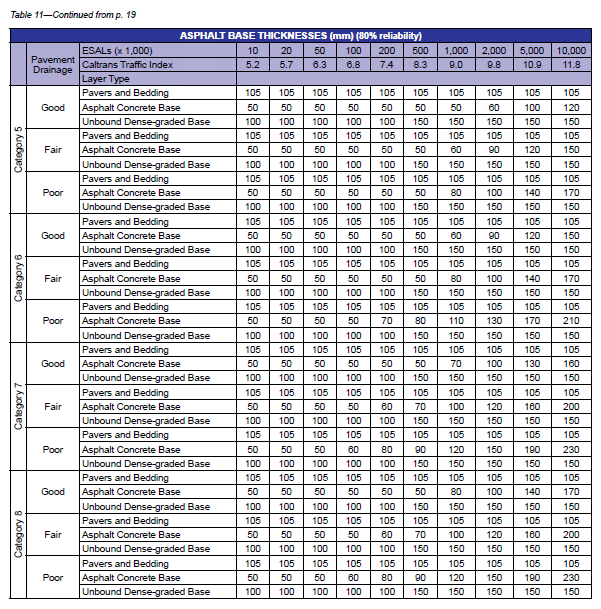
OTHER DESIGN CONSIDERATIONS AND CONSTRUCTION DETAILS
Guidance is also provided on proper detailing around utility structures, including edge detailing with sailor and soldier courses. Particular emphasis is given to drainage details for unbound aggregate and treated bases. This benefits pavement life and performance for all structural designs, and some details unique to interlocking concrete pavements are shown in Figures 7 and 8. For further details, design considerations, best practices and maintenance procedures designers are directed to the Tech Note series and detail drawings available at www. MasonryAndHardscapes.org. The designer is also encouraged to address how interlocking concrete pavement can contribute to sustainability through applying the Leadership in Energy and Environmental Design (LEED®) credit system. Additional information on LEED® credits can be found in Tech Note PAV-TEC-016–Achieving LEED® Credits with Segmental Concrete Pavement.
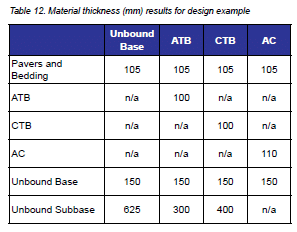
COMPUTERIZED SOLUTIONS
The preceding design example and most interlocking concrete pavement for parking lots and roads can be designed with “Interlocking Concrete Pavement Structural Design Program” that uses Excel-based software. The software is based on the ASCE 58-16 design standard and generates thickness solutions for unbound aggregate base, asphalt- and cement-treated, and asphalt concrete bases.
After a pavement structure has been designed, the user can project life-cycle costs by defining initial and lifetime (maintenance and rehabilitation) cost estimates. CMHA offers life cycle cost analysis (LCCA) software in Excel format. This software enables LCCA comparisons among interlocking concrete pavement, asphalt and concrete pavements. It can perform deterministic and probabilistic cost analyses. Design options with initial and maintenance costs plus discount rates can be examined for selection of an optimal design from a budget standpoint. Sensitivity analysis can be conducted on key cost variables on various base designs. For further information on both free software programs, contact CMHA members, CMHA offices or visit www.MasonryAndHardscapes.org.
GEOSYNTHETICS
Geotextiles, geogrids and cellular confinement systems are seeing increased use in pavements. Geotextile selection and use should follow the guidance provided in AASHTO M288. Geotextiles are placed over the top of the compacted soil subgrade and separate the soil from the base materials. These are recommended over silt and clay soils. Geogrids are sometimes used in very soft, wet and slowly draining soils. Cellular confinement systems filled with base materials and placed over the compacted soil subgrade have been used to reduce base thicknesses. Manufacturer’s literature should be consulted for guidance on reduction of base thickness given anticipated traffic and soil conditions.
RIGID PAVEMENT DESIGN WITH INTERLOCKING CONCRETE PAVERS
Rigid pavements consisting of Portland cement concrete (PCC) slabs distribute loads to the compacted soil subgrade through flexure, or bending action. In such pavements the load spreading is primarily a function of the thickness of the slab and the flexural strength of the PCC. Base materials are often placed under the slab to provide additional structural support and drainage.
When PCC slabs are used as a base under concrete pavers, the structural contribution of the concrete pavers is often ignored by designers. The following sections provide a design method that includes some structural contribution by concrete pavers and bedding materials over PCC slabs as well as over roller compacted concrete. This pavement assembly requires consideration of the bedding materials, prevention of bedding sand loss and avoiding discontinuities over slab joints. Detailing that addresses these aspects are also covered in the following sections.
BACKGROUND TO PCC PAVEMENTS
There are three main types of PCC pavement; jointed concrete pavements (JCP), jointed reinforced concrete pavements (JRCP) and continuously reinforced concrete pavements (CRCP). Although other types are used, this Tech Note will only address these three PCC systems. The differences among them are primarily in how environmental effects are controlled such as moisture change and temperature changes, including curing and environmental factors. These factors affect the reinforcement and jointing arrangements with little change in the slab thickness.
As concrete cures and dries, water in small pores within the cement creates surface tension. This force pulls the pore walls closer together causing the volume of the cement paste to shrink. This action reduces the entire paving slab size slightly. As the slabs are partially restrained by friction from the underlying base or soil subgrade, tensile stresses develop that can result in shrinkage cracks. The stress from shrinkage is proportional to the length of the section of pavement. To control the shrinkage it is therefore necessary to provide joints at sufficiently close centers to keep the shrinkage stresses below the tensile strength of the concrete. Alternatively, reinforcement can be used to increase the tensile strength of the pavement so that greater joint spacing can be used.
As concrete pavements heat up the slabs expand, and when they cool the concrete contracts. This movement results in closing and opening of the joints in the pavement. As expansion and contraction are proportional to the length of the slab, the movement range increases with greater joint spacing. Movement is also proportional to the temperature range, so this also requires consideration when designing the joints. Typically concrete can expand or contract by about 1/16 in. (1.5 mm) for every 10 ft (3 m) over an 80°F (27° C) temperature change. Pavement temperatures generally fluctuate over a wider range than air temperatures.
Thickness design for PCC pavements for low-speed roads and parking lots is typically done according to the 1993 AASHTO Guide or to local adaptations. Different equations are used for the design of rigid pavements than those for flexible pavements. The thickness of PCC pavements is determined to resist wheel loads imposed by the predicted traffic. Thickness depends on the soil conditions, the type of subbase, the edge conditions, the reliability requirements and the number of 18,000 lb (80 kN) ESAL repetitions. Some design considerations follow and thickness design is covered in greater detail later.
JOINTED CONCRETE PAVEMENT
In a jointed concrete pavement (JCP) the joints are placed at close centers so that curing shrinkage does not lead to random cracking, and that joint widths are restricted to acceptable limits. The joints may have load transfer devices such as steel dowel bars (doweled JCP), or the interlocking of the aggregate particles on each face of the joint may be sufficient to transfer the loads from one side of the joint to the other (plain JCP).
The joint spacing is dependent upon the thickness of the concrete. A general rule of thumb is that the joint spacing should not exceed thirty times the slab thickness and should in no case exceed 20 ft (6 m). Individual panels should generally have a length of no more than 1.25 times the width. For doweled joints the joint spacings are typically between 10 and 20 ft (3 and 6 m) with joint widths potentially up to 1/8 in. (3 mm). For plain joints, the joint spacings are typically 10 to 15 ft (3 to 4.5 m) with joint widths of up to 1/16 in. (1.5 mm). This type of pavement is the best solution as a base under interlocking concrete pavers.
JOINTED REINFORCED CONCRETE PAVEMENT
Jointed reinforced concrete pavements are designed with longitudinal and transverse reinforcement to accommodate the tensile stresses that arise during curing. This enables greater joint spacing to be achieved, but results in wider joint opening. As such, aggregate interlock cannot be relied upon and all joints require load transfer devices such as dowels. The reinforcement is typically located at about mid-depth in the slab so it does not increase the load capacity of the concrete section, As such, the same thickness of slab is required as for jointed concrete pavements. Joint spacings are typically 15 to 60 ft (4.5 to 18 m) with joint widths of up to 1/2 in. (13 mm). Intermediate joints are usually included to enable construction activities and to control warping of the slabs.
Warping occurs when there is a temperature difference between the top and the bottom of the concrete that causes it to curl. The intermediate joints are typically spaced at 10 to 20 ft (3 to 6 m) and include tie bars to keep the two sides of the joint from moving relative to each other. Large joint spacings can be problematic under pavers as joint movement reflects to the surface resulting in bedding sand loss and localized settlement and loosening of the pavers.
CONTINUOUSLY REINFORCED CONCRETE PAVEMENT
Continuously reinforced concrete pavements are designed with a greater amount of longitudinal reinforcement so that they can be constructed without transverse joints. The same thickness of slab is required as for jointed concrete pavements as the reinforcement does not increase the load capacity of the pavement. There is a general acceptance that transverse cracking will occur, however, the cracks are held tightly together by the longitudinal reinforcement. The cracks are initially widely spaced, but with full curing, subsequent traffic and temperature changes, the cracks may develop as closely spaced as 2 ft (0.6 m). Minor opening and closing of these joints are generally considered to accommodate the expansion and contraction of the pavement. Reinforcement in the transverse direction is generally similar to that in jointed reinforced concrete pavements. Longitudinal joints are constructed in a similar fashion to jointed reinforced concrete pavements. They may have tie bars or dowels depending on the pavement width. Excessive spacing of longitudinal movement joints may result in localized movement of the overlying pavers.
JOINTS
As described above, the joints in a concrete pavement control cracking from curing shrinkage and to permit movement caused by moisture and temperature changes. Joint locations should provide adequate load transfer across each from aggregate interlock or from load transfer devices. Joints are typically laid out in a rectangular grid pattern with joints meeting edges of the pavement at no less than 60 degrees. Joints should not deadend at another joint. They should be detailed to prevent ingress of moisture and infiltration of foreign mater.
There are three basic joint types that are formed during pouring or induced shortly afterwards. These are contraction joints, expansion joints and isolation joints. Each are described below and how they should be detailed when under interlocking concrete pavement.
CONTRACTION JOINTS
Contraction joints provide a release for tensile stress in the pavement as the concrete contracts during curing. When they are induced in the interior of a pour of concrete, they are often referred to as weakened plane joints as they cause a crack to occur in a defined position. In addition to being formed during pouring, weakened plane joints may be induced by early sawing, or by inserting crack-inducing plastic strips. Their placement controls where the tensile failure will occur so that the resulting cracks are in pre-defined positions, preventing random cracking. They can be oriented in the transverse and longitudinal directions relative to the pavement. The spacing of the joints is determined based upon the materials used, the thickness of the slab and the local environmental conditions, as described above. Load transfer devices are used when the joint opening is too wide to permit aggregate interlock. Contraction joints should be covered with minimum 12 in. (300 mm) wide woven geotextile strips to prevent bedding sand loss under concrete pavers.
EXPANSION JOINTS
Expansion joints perform in the same way as contraction joints but are also used to accommodate any longitudinal or transverse expansion of the pavement that exceeds the drying shrinkage. A compressible filler board absorbs any compressive stresses induced in the concrete by expansion. Where possible, their use is minimized with their most frequent location being at changes in the pavement construction and at intersections or other fixed structures in the pavement surface. In some cases the joint may also need to accommodate lateral movement. Expansion joints should generally be carried through the paver surfacing with the installation of edge restraints on either side.
ISOLATION JOINTS
Isolation joints are used in locations where movements in the pavement are to be isolated from an adjacent feature. They may be used against a building, a utility structure or other feature where vertical and horizontal movement could impose unwanted load into that feature. They are normally formed by including a compressible filler board without any load transfer devices. Isolation joints do not generally experience significant movement and they should be covered with a woven geotextile to prevent bedding sand loss.
ROLLER COMPACTED CONCRETE BACKGROUND
Roller compacted concrete (RCC) behaves in a similar fashion to jointed concrete pavement and may be used as an alternative base under interlocking concrete pavement. Fresh RCC consists of a semi-dry concrete spread through a modified asphalt paving machine. PCC aggregates are used in the mix and the final strength is similar paving quality concrete.
Mix designs are prepared in the laboratory to determine compressive strength and maximum density. Compressive strengths of 3,000 to 5,000 psi (20 to 35 MPa) may be specified. Compaction is initially done by the paving machine and finally by rollers until the target density is achieved. This is typically 98% of modified Proctor density. RCC may be placed without joints, or joints can be induced on a regular grid. When joints are not planned, the roller compacted concrete develops a network of narrow cracks during curing. The curing shrinkage is far less than for PCC pavements so the joints and cracks transfer loads by aggregate interlock. Design thicknesses are similar to those for PCC pavements.
TRAFFIC
The AASHTO equations for pavement design express serviceability loss as a measure of pavement damage. The damaging effect of axles is different between the flexible and the rigid pavement equations. This is reflected in the AASHTO design method by having a different flexible ESAL values to rigid ESAL values, however the difference is not considered to be significant for design of interlocking concrete pavements over concrete.
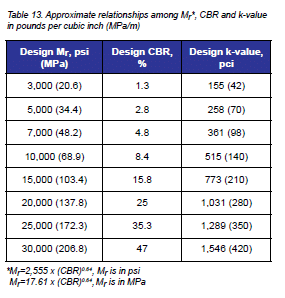
SOIL SUBGRADE SUPPORT
The AASHTO design method for rigid pavements uses the soil subgrade property known as the Modulus of Subgrade Reaction or k-value. This value is determined using a plate load test that is different than those described above in the flexible pavement section. The test is described in ASTM D1194 or AASHTO T-235. It involves placing a 30 in. (0.76 m) diameter rigid plate on the subgrade and measuring the deflection of the soil as the load on the plate is gradually increased. The k-value is determined as the pressure divided by the deflection at during certain points in the test. The test is rarely carried out and alternative means are generally used to establish the design value.
The design k-value is considered at the underside of the concrete, and includes the effects of any subbase layers. The AASHTO method also includes seasonal changes of subgrade strength and the proximity of rock to the surface to develop a composite k-value for design. This provides a wide range of k-values although the designed thickness has low sensitivity to this property. As such, the design charts in this Tech Note are simplified to use an approximate relationship between the design resilient modulus (Mr) and the k-value. The design values are listed in Table 13. The values stated assume no subbase is present and that the depth to a rigid rock layer exceeds 10 ft (3 m). Where soils are known to be prone to pumping under concrete pavements, a minimum of 4 in. (100 mm) of compacted aggregate subbase material over the subgrade is recommended prior to casting the concrete. This thickness is also recommended with soils with an Mr < 7,000 psi (48 MPa) or CBR < 5%.
PAVEMENT MATERIALS
Most states, provinces and municipalities have material and construction standards for concrete pavements. However, material requirements vary among jurisdictions, particularly material strengths. The design tables on the following pages with rigid pavement base layer thicknesses are based upon typical values encountered in many standards.
There are two properties used in the AASHTO design method to characterize PCC pavements; flexural strength and the elastic modulus. Typically pavement quality concrete is specified with a flexural strength, although compressive strength is occasionally substituted. The flexural strength should be determined using beam specimens loaded at third points as described in ASTM C78 or AASHTO T-97. If compressive strength is the only requirement available, the designer can use Table 14 to provide an approximate correlation. The elastic modulus of concrete is rarely specified and so typical relationships to flexural or compressive strengths are required as provided in Table 14. The AASHTO design equation is based upon the average value of flexural strength, which will be slightly higher than the specified value. When PCC is used as a base under concrete pavers it is usually not necessary to include an air entraining agent. The pavers provide protection against damage from frost action.
Reinforcement is not considered in the AASHTO design equation for determining the PCC pavement thickness. However, the type of reinforcement is important in determining the required bar sizes and centers and the spacing of joints. Typically, reinforcing bars and tie bars are Grade 60 deformed bars in size numbers #4, #5 or #6. However, Grade 40 steel may be used. As jointed concrete pavement is the preferred base condition, no additional guidelines are provided for determining the size and spacing of reinforcement. Dowel bars are typically Grade 60 in sizes ranging from 1/2 to 11/4 in. (13 to 32 mm). Table 15 sets out typical recommendations for dowel bars recommended by the American Concrete Institute. All dowel spacings are 12 in. (300 mm) on center.
Joint filler board is used in expansion joints and isolation joint to absorb any compression as the adjacent slabs move or expand. There are several different types including foam and bitumen impregnated fiber board. The thickness is selected dependent on the anticipated movement. Joint sealant is used to prevent the ingress of moisture and intrusion of foreign matter into joints. It may not be required on all joints when the concrete is exposed at the surface of the pavement if the movement range is small and if the lower layers are not moisture susceptible. When jointed concrete pavement is used under pavers the sealant may be left off if the joints are covered by geotextile. Sealant is recommended for joints with wider spacings.
Woven geotextiles are recommended to cover the joints and cracks in the PCC base to prevent bedding sand loss. Since they are manufactured from plastics such as polypropylene and polyester, the materials are stable and resistant to many chemicals encountered in the ground, and also to the deteriorating effects of sunlight. Woven geotextiles are preferred for use directly under the bedding sand as they maintain their integrity under loads exerting abrasion on the concrete. The important property in geotextiles for preventing sand loss is the apparent opening size or AOS. Woven geotextiles with an apparent opening size of 0.300 mm to 0.600 mm are generally suitable. As noted earlier geotextiles are applied to joints in minimum 12 in. (300 mm) wide strips.
STRUCTURAL DESIGN PROCEDURE
The following structural design procedure is for roads and parking lots. PCC pavements are designed using a simplified version of the method in the AASHTO 1993 Guide. These pavement sections were then analyzed using mechanistic analysis to determine the critical stresses. The pavements were also analyzed considering a concrete paver surface to distribute the loads to a larger area on top of the concrete. The pavements were reduced in thickness incrementally until the same critical stresses were achieved in the concrete. The results of the analyses are presented in the tables. All designs are minimum 31/8 (80 mm) thick concrete pavers in a herringbone pattern. Bedding materials are sand or sand-asphalt (bitumen-setting bed). Tech Note PAV-TEC-017–Bedding Sand Selection for Interlocking Concrete Pavements in Vehicular Applications provides guidance on testing and selecting bedding sands. Tech Note PAV-TEC-020–Construction of Bituminous-sand Set Interlocking Concrete Pavement provides guidance on this installation method.
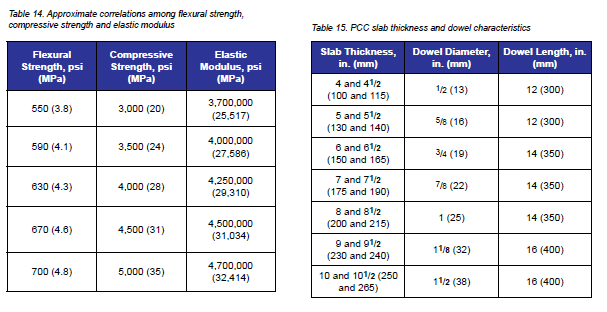
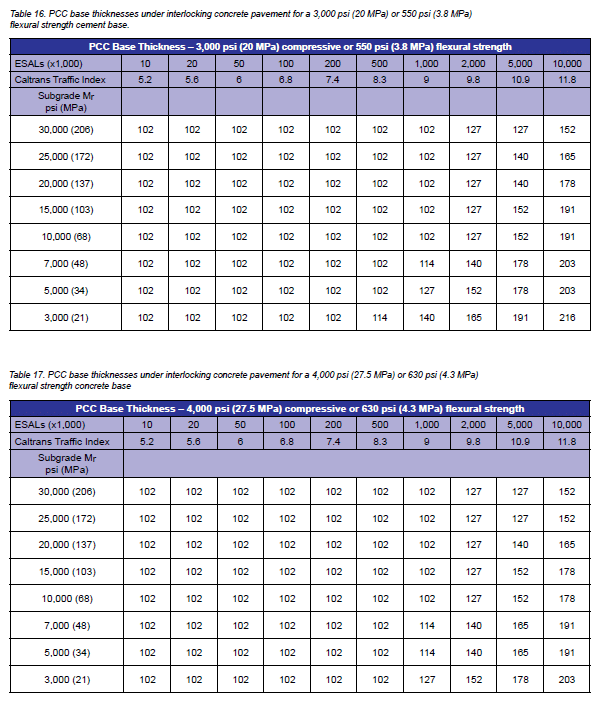
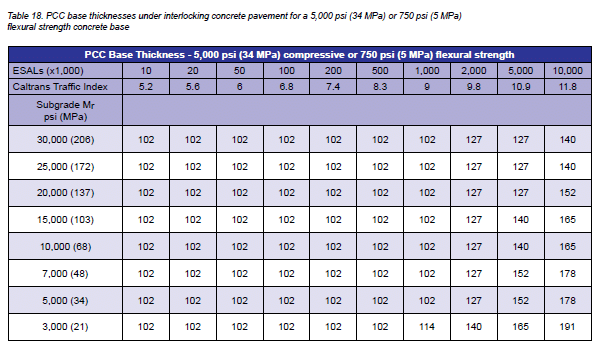
STRUCTURAL DESIGN TABLES
Tables 16, 17, and 18 establish the PCC base thickness design solutions. Depending on the soil subgrade strength (Mr) and ESALs. The recommended minimum thickness of PCC base is 4 in. (100 mm) at and below 1,000,000 ESALs, and 5 in. (125 mm) above 1,000,000 ESALs.
Use the following steps to determine a pavement thickness:
- Compute design ESALs or convert computed TIs to design ESALs or use the recommended default values given in Table 1 as for flexible base design.
- Characterize the soil subgrade strength from laboratory test data. If there is no laboratory or field test data, use Tables 3, 4 and 5 to estimate Mr.
- Select the appropriate table (16, 17 or 18) depending on the compressive strength of the concrete base.
- Determine the required PCC base thickness. Use Mr for design subgrade strength and design ESALs in the selected tables.
EXAMPLE SOLUTION AND RESULTS
For a given site where the soils are ML, it is assumed that an aggregate subbase will be used to provide a working platform and to protect the pavement from pumping related distress.
- Estimate design load: 840,000 ESAL. Interpolate between 500,000 and conservatively select 1,000,000 when using Tables 16, 17 or 18.
- Characterize subgrade Mr: 4,500 psi (31 MPa) from previous example. Conservatively select 5,000 psi (35 MPa) on Tables 16, 17 or 18.
- Determine concrete strength: Consider 3,000 psi (21 MPa) and 4,000 psi (27.5 MPa) options on Tables 16 and 17.
- Determine base thickness requirements: the thickness required for 3,000 psi (20 MPa) concrete is 5 in. (125 mm) and for 4,000 psi (28 MPa) concrete is 41/2 in. (115 mm).
The final cross section design is shown in Figure 8 on page 28 with 31/8 in. (80 mm) thick concrete pavers and a 1 in. (25 mm) thick bedding sand layer over 41/2 in. (115 mm) of 4,000 psi (27.5 MPa) PCC base and 4 in. (100 mm) compacted aggregate subbase since the soil Mr < 7,000 psi (48.2 MPa) which is CBR < 5%. As on flexible bases, concrete pavers on rigid bases should not exceed an aspect ratio (length divided by thickness) of 3. Additionally, the concrete slab is jointed at 10 ft (3 m) centers and dowels are 1/2 in. (13 mm) diameter. The joints will be covered with a strip of woven geotextile, minimum 12 in. (300 mm) wide, to prevent bedding sand loss.
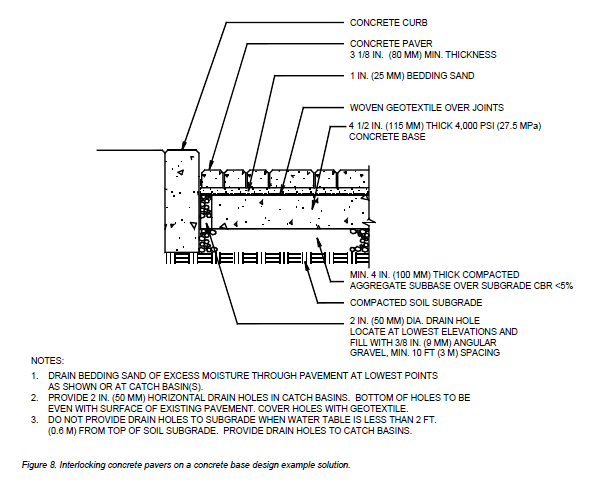
REFERENCES
- AASHTO 1993. American Association of State Highway and Transportation Officials, Guide for Design of Pavement Structures, Washington, D.C., USA.
- ASCE 2016. American Society of Civil Engineers, Structural Design of Interlocking Concrete Pavement for Municipal Streets and Roadways. ASCE/T&DI 58-16, Reston, Virginia, USA.
- Rada 1990. Rada, G.R., Smith, D.R., Miller, J.S., and Witczak, M.W., “Structural Design of Concrete Block Pavements,” American Society of Civil Engineers Journal of Transportation Engineering, Vol. 116, No. 5, September/ October.
- Shackel 1979.Shackel, B., “A Pilot Study of the Performance of Block Paving Under Traffic Using a Heavy Vehicle Simulator,” Proceedings of a Symposium on Precast Concrete Paving Block, Johannesburg, South Africa.
- Shackel 1980. Shackel, B., “An Experimental Investigation of the Roles of the Bedding and Joint Sand in the Performance of Interlocking Concrete Block Pavements,” Concrete/Beton, No. 19.
- Shackel 1980. Shackel, B. “Loading and Accelerated Trafficking Tests on Three Prototype Heavy-Duty Industrial Block Pavements,” National Institute for Transport and Road Research, CSIR, Pretoria, South Africa, Technical Report 12.
Figure 1 photo credit iStock.com. Figures 5 and 6, Tables 5, 6 and 7 are copyright © 2010 ASCE. Used with permission.